Lasers in fluorescence microscopy
Today’s high-end fluorescence microscopy is unthinkable without lasers to elicit fluorescence exactly where and how it is needed. Reason enough to take a closer look at these sophisticated light sources.
what’s so exciting?
Let’s start with a rather obvious fact: Optical microscopy requires light. The specimen has to be illuminated in some way to reveal information on its shape and structure. In classical widefield microscopy, the demands on the light are quite simple: it has to be bright enough to reveal sufficient detail. Standard widefield microscopes usually work with mercury or xenon gas-arc lamps or LEDs and the light is evenly distributed over the specimen.
For confocal fluorescence microscopy, however, things are more complex, and in stimulated emission depletion (STED) microscopy even more so. Which is the reason why at abberior we devote particular attention to the laser topic.
But before we go into more detail, let’s first see what requirements confocal microscopy places on the light. For one, only a very small part of the sample is investigated at a time, and the light needs to be focused on precisely this spot. For another, fluorescence microscopy relies on fluorescent molecules – called fluorophores – in the studied sample, hence its name. When irradiated with light of a defined wavelength, these fluorophores emit light of a different (higher) wavelength. (The wavelength determines the light’s color: light with a wavelength of around 450 nm, for instance, is blue and light of approximately 700 nm is red; green and yellow lie in between the two). Every fluorophore has its own specific spectrum of excitation – where it takes up the light’s energy – and emission – where it emits light of its own. For this to work effectively, the excitation light has to be of exactly the right wavelength and highly focusable. Which is why you need a laser.
Everyone knows what a laser is, right?
Laser stands for “light amplification by the stimulated emission of radiation”, which describes the way it generates light. Even kids – and not only those having watched Star Wars – will be able to tell you that lasers produce powerful light beams in a multitude of colors.
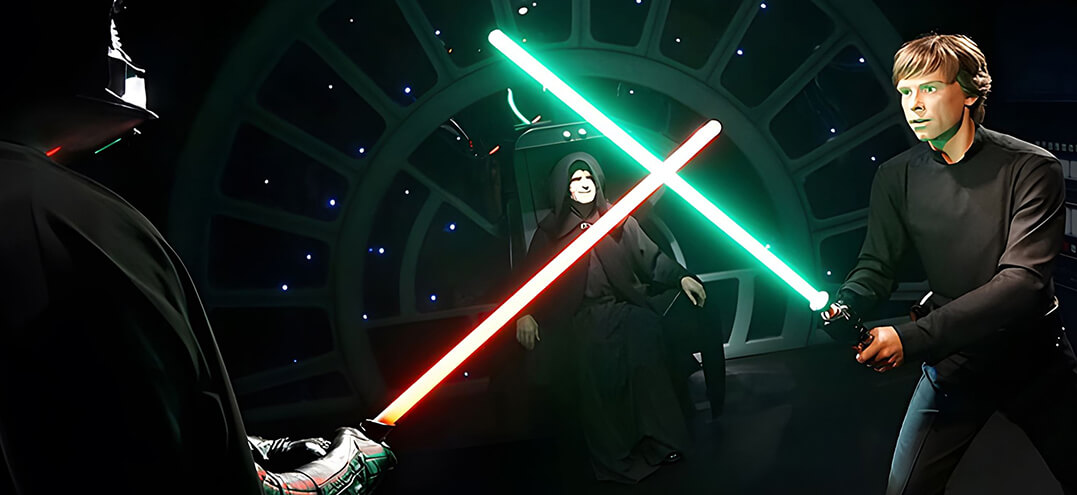
Figure 1. Darth Vader and Luke Skywalker with laser lightsabers at Madame Tussauds. Due to their high power, lasers spark imagination and in science fiction often serve as weapons. Image: Mirko Toller, Wikimedia Commons, CC BY 2.0
And this description is in fact pretty close to the actual physical definition, stating that a laser is a light source generating monochromatic, coherent, and unidirectional irradiation.
Ehm, come again?
All right, one by one: Laser light is
- monochromatic: it consists of only one wavelength or a narrow range of wavelengths, equaling a specific color.
- coherent: it has one wave form and frequency
- unidirectional: all light waves go in the same direction, creating a beam
These are all properties unavailable from classical light sources, which is why the development of lasers in the 1960s incited an avalanche of new technologies exploiting the new power of laser light.
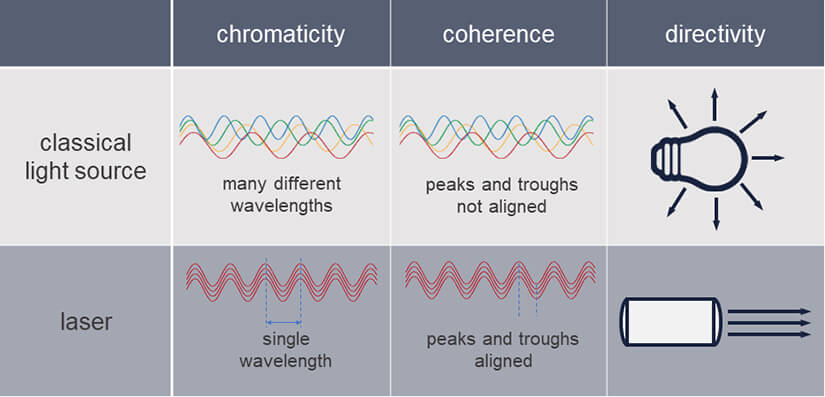
Table 1. Comparison of classical light sources and lasers. Lasers generate light that is monochromatic, coherent, and unidirectional.
But there is not “the one laser”. Many different types of lasers have been developed over time and they come with quite distinct properties, perfectly shaped for various applications all around us – from laser pointers to printers, scanners, welding lasers and lasers for medical or scientific applications, to name just a few. They are usually classified by their gain medium, which is the actual source of their light: There are gas lasers, liquid lasers, solid-state lasers, and semiconductor or diode lasers.
The power to excite
In microscopy, lasers are most commonly used to excite fluorophores. A typical excitation laser has a power output in the range of a few milliwatts. For a long time, one of the most commonly used excitation lasers in fluorescence microscopy was the Argon-ion laser. This type of laser produces light of a number of wavelengths conveniently matching the absorption spectrum of various fluorescent dyes. This allows for the excitation of multiple dyes simultaneously, facilitating multicolor imaging.
Another popular laser used in fluorescence microscopy is the Helium-Neon laser (HeNe). HeNe lasers produce a single fixed wavelength of light (usually red), which makes them a stable and easy-to-use option.
In recent years, compact and affordable diode lasers have replaced many other types. They offer several advantages, including low power consumption, high efficiency, and ease of use. Moreover, they can be operated in pulsed mode, which is of great advantage for fluorescence microscopy. Pulsing means that the laser light is not emitted continuously (as with continuous-wave (cw) lasers), but as short, periodic pulses, which are usually a few 100 picoseconds long with a pause in between of a few ten nanoseconds. This way, the power is concentrated on the short pulses, greatly alleviating the energy burden on a specimen, a critical prerequisite for live cell imaging as both photobleaching and phototoxicity are reduced. Pulsed lasers are also a prerequisite for fluorescence lifetime imaging, which is based on measuring the time between a light pulse and the arrival of photons.
abberior’s STEDYCON, FACILITY, and INFINITY microscopes are all equipped with diode lasers for excitation that get on with a power below 100 microwatts.
White light lasers – a solution to all problems?
Most recently, fiber-based pulsed white light lasers have also gained traction. Their biggest plus is their versatility: They do not emit at a single or few wavelengths, but over the full spectrum. But wait – did you pay attention? White lasers seem to violate the definition of lasers as their light is not monochromatic. However, they generate white light by a so-called non-linear optical process from monochromatic light. And their light is unidirectional and coherent, in line with the definition. So we can speak of white lasers as lasers, after all.
With a tunable filter any desired wavelength can be selected from a white laser. Sounds good, doesn’t it? Well, only up to this point, for cost and complexity for both the laser and the required tunable filters are high. And when the only laser breaks down, the whole microscope is instantly unusable.
In superresolution microscopy, white lasers have another pivotal drawback: The fact that a wide range of wavelengths is emitted from the same source means that chromatic aberrations cannot be compensated for all wavelengths and objective lenses, which significantly reduces image quality.
The power to deplete
Stimulated emission depletion (STED) microscopy introduces a new level of complexity to the topic of lasers as here fluorophores are not only excited but also deexcited. The light to shut down undesired fluorescence is delivered by a STED laser, and the requirements for this job are quite distinct from those for excitation. Most importantly, STED lasers must deliver much higher intensity light and allow pulsed operation. The former guarantees that fluorescence is de-excited efficiently in the area of the STED beam while the latter ensures that the energy is focused in time, precisely right after the excitation pulse when it achieves maximum effect on the excited dye. Any photons arriving at the sample outside this narrow time slot are entirely useless and only do harm by bleaching fluorophores or damaging the sample – which is why cw STED lasers are not a practical option (Fig. 2).
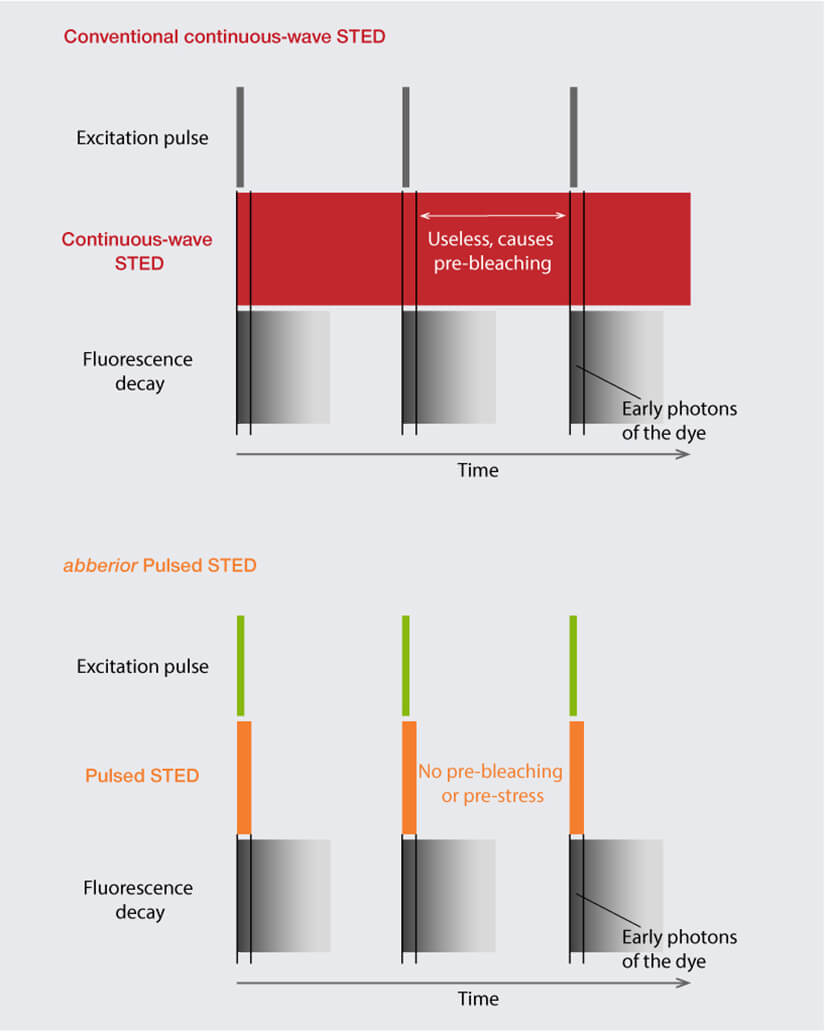
Figure 2. Comparison of cw STED with abberior’s Pulsed STED. cw STED lasers continuously shine light on the sample, with a high portion of energy wasted as it is delivered outside the time slot where fluorescence occurs, causing avoidable photobleaching. abberior’s Pulsed STED laser focuses the energy in time right after the excitation pulse, achieving maximum effect on the excited fluorophores.
In the past, pulsed titanium-sapphire lasers were often used for STED microscopes, but compact, reliable and affordable fiber-lasers have made them largely obsolete for this application. The STED lasers installed in abberior microscopes are pulsed diode lasers with powers between 1 and 3 watts.
Every superresolution technique poses its own demands
Superresolution techniques like PALM and STORM, the most important types of single molecule localization microscopy (SMLM), in turn come with other requirements for their lasers. Since they are essentially wide-field techniques, the excitation light is constantly spread over the full field of view. This requires high-power, typically fiber-based lasers, in the range of 1 to 5 watts to achieve the local power density required for excitation. If you want to learn more about SMLM, we recommend this article.
MINFLUX is the single fluorescence microscopy method that reaches molecular resolution. It also holds the temporal resolution record in the field. One would expect this high-end microscopy technique to need particularly sophisticated lasers. The requirements indeed differ from those posed by STED but are not necessarily stricter. Commonly, MINFLUX instruments use a diode laser with a power of several hundred milliwatts.